The Bone Advantage: Unlocking Athletic Potential Through Skeletal Development
- Fitfty
- 6 days ago
- 11 min read
Structural Excellence: The Skeletal Science Every Athlete Should Understand
Article 2 of 4 in “The Strength Paradox” series

When we think of athletic performance, muscles often steal the spotlight. The bulging biceps of a weightlifter or the sinewy legs of a marathon runner capture our attention.
Yet beneath this muscular showcase lies the true architectural framework of human movement — our skeleton. Bones provide the rigid structure against which muscles pull, creating a biomechanical marvel capable of extraordinary feats. However, the role of skeletal development in athletic potential remains largely underappreciated by the general public and many athletes alike.
This article explores the fascinating world of bone tissue and its critical importance to athletic performance. From the embryonic formation of our skeletal structure to its adaptation throughout life, we’ll investigate how this remarkable tissue supports — and potentially limits — human physical capability.
The Science of Bone: More Than Just Calcium
Bone is a dynamic, living tissue constantly undergoing a process of renewal. Far from being the inert calcium scaffolding depicted in anatomy textbooks, bone tissue hosts a complex cellular ecosystem.
The Cellular Architecture
Three main cell types govern bone’s continuous remodeling:
Osteoblasts: These bone-building cells synthesize and secrete the organic matrix of bone (primarily type I collagen) which later becomes mineralized with calcium hydroxyapatite crystals.
Osteoclasts: These cells function as bone’s demolition crew, breaking down old or damaged bone tissue through a process called resorption.
Osteocytes: Former osteoblasts that become embedded within the bone matrix, these cells form a communication network throughout the skeleton, sensing mechanical forces and orchestrating the remodeling process.
The balance between formation (osteoblasts) and resorption (osteoclasts) determines bone mass and strength. This equilibrium shifts throughout life, favoring formation during growth years and tipping toward resorption in later decades.
Composition and Structure
Bone tissue combines organic and inorganic components in an elegant compromise between strength and flexibility:
Organic matrix (35%): Primarily collagen fibers providing tensile strength and flexibility
Inorganic minerals (65%): Predominantly calcium hydroxyapatite crystals conferring compression resistance
Water and cells: Occupying spaces within the matrix
Structurally, bone exists in two forms:
Cortical (compact) bone: The dense outer layer that provides structural integrity
Trabecular (cancellous) bone: The honeycomb-like interior structure optimized for weight-bearing while minimising mass
This sophisticated architecture allows bones to be simultaneously lightweight yet remarkably strong. The femur (thigh bone), for instance, can withstand compressive forces 5–6 times body weight during normal walking and up to 10 times body weight during intense activities.
Newtonian Physics in Skeletal Biomechanics
The principles of classical mechanics describe how bones function as levers and load-bearing structures during movement.
Levers and Mechanical Advantage
Human bones operate as mechanical levers with joints serving as fulcrums. According to Newton’s laws of motion, the arrangement of these levers determines mechanical advantage — how efficiently force applied by muscles translates into movement.
Consider the elbow joint:
The forearm serves as a third-class lever where the effort (biceps) lies between the fulcrum (elbow joint) and the load (hand)
This arrangement sacrifices force for speed and range of motion
An athlete with longer forearm bones relative to biceps insertion gains velocity advantage but loses force production
By contrast, the ankle joint in jumping:
Functions as a second-class lever with the ball of the foot acting as the fulcrum
This arrangement provides greater mechanical advantage for force production
Athletes with specific bone proportions in the foot and ankle may possess natural advantages in explosive movements
Stress and Strain
When forces act upon bone, two physical properties become critical:
Stress: The internal forces developed within the bone in response to applied loads, measured as force per unit area
Strain: The resulting deformation or change in dimension
Bones exhibit viscoelastic properties — they deform under stress but return to their original shape when the stress is removed, provided the force doesn’t exceed the bone’s yield point. Beyond this threshold, permanent deformation or fracture occurs.
Understanding these principles helps explain why:
Gradual training allows bones to adapt to increasing loads
Sudden excessive forces can cause catastrophic failure (fractures)
Different skeletal structures among individuals may predispose them to excel in particular sports
Embryonic Development and Growth: Building the Framework
The journey of bone development begins remarkably early in embryonic life through two distinct processes.
Ossification Pathways
Intramembranous ossification: Flat bones like the skull develop directly from mesenchymal tissue, with stem cells differentiating into osteoblasts and forming bone without a cartilage intermediate.
Endochondral ossification: Long bones develop from cartilage models that are gradually replaced by bone tissue. This process begins during embryonic development but continues well into adolescence at growth plates — specialized zones where new bone formation drives longitudinal growth.
The timing and rate of ossification follow genetic programs but remain sensitive to environmental factors, including nutrition and mechanical stimuli.
Critical Growth Periods
Bone development follows a predictable timeline with several critical periods:
Fetal development: Basic bone architecture is established
Early childhood: Rapid growth and mineral acquisition
Adolescent growth spurt: Peak velocity of bone elongation (earlier in females than males)
Late adolescence: Consolidation and peak bone mass attainment
The adolescent growth spurt deserves special attention from a sports perspective. During this period:
Bone growth outpaces muscle adaptation, creating vulnerability
Mechanical loading through appropriate exercise enhances bone mineral density
Excessive training or nutritional deficiencies can disrupt normal development
These critical periods represent both opportunity and risk — chance to optimise skeletal development for athletic performance but also vulnerability to developmental disruption through improper training or nutrition.
Bone Adaptation to Mechanical Loading
Bones adapt to the mechanical demands placed upon them — a principle first articulated by Julius Wolff in the 19th century (Wolff’s Law) and refined by modern research.
Mechanotransduction: From Force to Cellular Response
When bone experiences mechanical loading:
Fluid flow within the canalicular network surrounding osteocytes creates shear forces
Osteocytes detect these forces through specialized cell membrane proteins
Biochemical signaling cascades are initiated
Bone remodeling processes are adjusted — formation increases in regions experiencing greater strain while resorption dominates in less-stressed areas
This elegant feedback system allows bone to continuously optimize its structure according to mechanical demands.
Training Effects on Bone
Different training modalities affect bone distinctively:
Impact/weight-bearing activities (running, jumping): Generate high-magnitude forces that stimulate significant bone formation, particularly at sites of impact
Resistance training: Creates tension through muscle pull on attachment sites, strengthening both the bone’s mineral content and architectural organization
Swimming/cycling: Provide limited bone-strengthening benefits due to reduced loading (explaining why elite swimmers often have lower bone density than runners)
Critical factors determining bone’s adaptive response include:
Strain magnitude: Higher forces (within physiological limits) drive greater adaptation
Strain rate: Rapid loading is more osteogenic than slow loading
Strain distribution: Novel loading patterns stimulate greater adaptation than repetitive, identical forces
Recovery periods: Allow the cellular machinery of adaptation to work effectively
The practical implication? Athletes across disciplines benefit from including some form of impact or resistance training to optimise skeletal health and performance, even if their primary sport involves limited bone loading.
Male vs. Female Skeletal Differences
Sexual dimorphism in skeletal structure significantly influences athletic performance differences between males and females.
Structural Variances
Key differences include:
Size and mass: Male skeletons average 15% larger with greater bone mineral density
Proportions: Males typically have broader shoulders relative to hips; females wider pelvises relative to shoulders
Joint geometry: Differences in specific joint angles (e.g., female Q-angle at the knee) affect biomechanics
Bone architecture: Males generally develop thicker cortical bone and more robust trabecular networks
These differences emerge during puberty under the influence of sex hormones — testosterone driving greater periosteal expansion in males, estrogen limiting periosteal growth but enhancing endosteal bone formation in females.
Performance Implications
These skeletal differences contribute to performance variations:
The male advantage in upper body strength partly reflects advantageous lever arms in the shoulder complex
Female pelvic structure offers both advantages (lower center of gravity enhancing stability) and challenges (altered knee alignment potentially increasing injury risk)
Differences in bone mass partially explain disparities in power generation capabilities
Importantly, these population-level differences show considerable individual variation. Many female athletes possess skeletal characteristics that enable exceptional performance even in traditionally male-dominated strength events.
Bone Disorders and Athletic Performance
Various bone conditions can significantly impact athletic potential and performance safety.
Common Bone Pathologies
Athletes should be aware of several key conditions:
Stress fractures: Microdamage accumulation exceeding the bone’s repair capacity, common in endurance athletes and often related to training errors or nutritional deficiencies
Osteoporosis/osteopenia: Reduced bone mineral density increasing fracture risk, particularly concerning in female athletes with menstrual dysfunction (part of the Female Athlete Triad)
Osteitis pubis: Inflammation at the pubic symphysis common in sports involving kicking, cutting movements, or repetitive pelvic stress
Avulsion fractures: Particularly in adolescents where muscles pull bone fragments away at attachment sites during rapid movements
Osteochondritis dissecans: Separation of cartilage and underlying bone, often at the knee or ankle, potentially leading to joint damage
Special Populations
Some athletes face unique skeletal considerations:
Young athletes: Still developing skeleton requires carefully programmed training to avoid growth plate injuries while capitalizing on development opportunities
Female athletes: Need specific attention to bone health through adequate energy availability, calcium/vitamin D intake, and appropriate loading patterns
Masters athletes: Must compensate for natural age-related bone loss with targeted training and nutrition
For all athletes, bone health represents a foundation of performance that, once compromised, can be difficult to fully restore.
Optimising Bone for Performance: Practical Applications
Athletes and coaches can leverage scientific understanding to develop bone-conscious training approaches.
Training Protocols
Evidence-based recommendations include:
Progressive overload: Gradually increasing forces applied to bones allows adaptive responses without excessive damage
Varied loading patterns: Incorporating multidirectional movements challenges bone in novel ways, enhancing adaptation
Impact periodization: Alternating high-impact training with recovery periods optimizes bone formation while minimizing injury risk
Strategic resistance training: Compound movements creating tension through key skeletal regions develop both muscle and bone
For sport-specific applications:
Runners benefit from hill training and plyometrics in addition to flat-surface work
Swimmers should incorporate land-based resistance training to compensate for limited skeletal loading in water
Team sport athletes need multidirectional movement patterns challenging the skeleton across various planes
Nutritional Support
Optimizing bone health requires specific nutritional attention:
Calcium: 1000–1300mg daily (higher end for adolescents and female athletes)
Vitamin D: 600–2000 IU daily with blood levels monitored
Protein: 1.6–2.2g/kg body weight daily supporting both muscle and bone matrix development
Energy availability: Sufficient calories preventing hormonal disruptions that compromise bone health
Micronutrients: Adequate magnesium, vitamin K, zinc, and other cofactors for bone metabolism
The integration of targeted training and nutrition creates a powerful synergistic effect on bone development and maintenance.
Is Bone the Limiting Factor in Human Strength?
We arrive at a fascinating question — does our skeletal structure ultimately constrain human strength potential?
Theoretical Limits
From a materials science perspective:
Healthy human femurs can withstand compressive forces of approximately 1,700–2,500 pounds
The tensile strength of cortical bone approaches 20,000 pounds per square inch
These values far exceed forces generated in most athletic endeavors
However, several considerations suggest the skeleton does indeed impose certain limits:
Lever disadvantages: Human skeletal proportions often sacrifice force for mobility and range of motion
Joint integrity: Connective tissues stabilizing joints typically fail before bones reach their mechanical limits
Safety margins: Evolutionary pressures favor bones strong enough for survival demands but not excessively heavy
Comparative Analysis
Examining our closest relatives provides enlightening context:
Chimpanzees possess shorter limbs creating more favorable power-generating lever systems
Their bone mineral density exceeds human values by 25–30%
This partly explains their ability to generate approximately 1.5 times the relative pulling force of humans
Yet humans developed specialised adaptations:
More efficient bipedal locomotion
Enhanced fine motor control
Superior throwing mechanics
These trade-offs reflect our evolutionary history, where endurance, tool use, and versatility offered greater survival advantages than raw strength.
Modern vs. Ancient Human Skeletons
Archaeological evidence reveals interesting contrasts between contemporary and ancient human skeletons:
Upper Paleolithic humans (50,000–10,000 years ago) typically had 20–30% greater bone mass and density than modern populations
Agricultural revolution populations (beginning ~10,000 years ago) show marked decline in bone robusticity with narrower, more gracile structures
Industrial-age skeletons continue this trend with further reductions in bone strength indicators
This suggests modern humans indeed possess comparatively weaker bones than our ancestors. Contributing factors likely include:
Reduced habitual physical activity
More processed diets lower in key minerals
Less environmental stress demanding skeletal robusticity
However, contemporary elite athletes often develop bone characteristics resembling pre-agricultural humans through intensive training and optimized nutrition — demonstrating our skeleton’s remarkable adaptability when properly challenged.
Conclusion: The Skeletal Foundation of Athletic Excellence
Our journey through the science of bone reveals a tissue of extraordinary complexity and adaptability — one that deserves far greater attention in athletic development programs. While muscles may execute movement and cardiorespiratory systems fuel activity, the skeleton provides the essential framework upon which athletic performance is built.
The evidence suggests that while bone does impose certain constraints on human physical capability, these limits remain theoretical for most athletes. Far more commonly, suboptimal skeletal development or maintenance represents an untapped reservoir of potential performance enhancement.
By understanding the principles governing bone adaptation and implementing targeted training and nutritional strategies, athletes can develop skeletal systems capable of withstanding greater forces, transferring energy more efficiently, and supporting higher performance levels across virtually all sports disciplines.
Perhaps most importantly, this approach promotes career longevity and health beyond competition — reminding us that in athletics, as in architecture, excellence begins with building upon the strongest possible foundation.
Next Up:
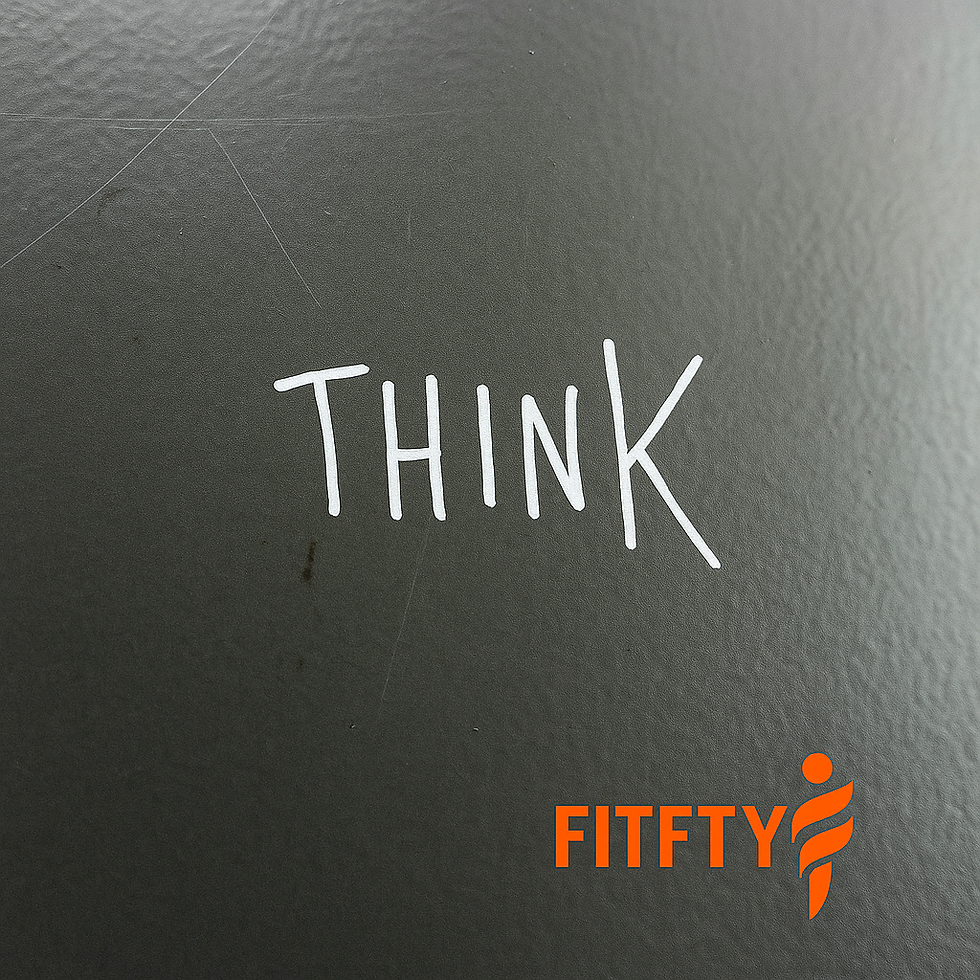
🔍 Explore More from Fitfty
Because strength isn’t a moment — it’s a movement.
9-Part Series | Get strength-ready from the ground up
Joints, bones, tissues, hormones, nutrition, mindset — this series is the blueprint your body’s been waiting for.
Series Menu:
The Strength Paradox: Why Everything You Know About Building Your Body is About to Change
2 — The Bone Advantage: Unlocking Athletic Potential Through Skeletal Development
3 — Muscle Metamorphosis: The Science of Contractile Tissue Transformation
4 — The Unified System: Bridging Science and Application in Strength Development
📚 References
Allen MR, Burr DB. (2023). Bone modeling and remodeling. In: Basic and Applied Bone Biology. Academic Press, 85–94.
Bass SL, Saxon L, Daly RM, et al. (2022). The effect of mechanical loading on the size and shape of bone in pre-, peri-, and postpubertal girls: a study in tennis players. Journal of Bone and Mineral Research, 17(12), 2274–2280.
Burr DB, Allen MR. (2019). Basic and Applied Bone Biology. Academic Press.
Crockett JC, Rogers MJ, Coxon FP, et al. (2021). Bone remodelling at a glance. Journal of Cell Science, 124(7), 991–998.
Frost HM. (2003). Bone’s mechanostat: a 2003 update. The Anatomical Record Part A, 275(2), 1081–1101.
Haapasalo H, Kontulainen S, Sievänen H, et al. (2020). Exercise-induced bone gain is due to enlargement in bone size without a change in volumetric bone density: a peripheral quantitative computed tomography study of the upper arms of male tennis players. Bone, 27(3), 351–357.
Hind K, Burrows M. (2022). Weight-bearing exercise and bone mineral accrual in children and adolescents: a review of controlled trials. Bone, 51(1), 81–101.
Pountos I, Giannoudis PV. (2023). Biology of bone healing and regeneration. In: Principles of Regenerative Medicine. Academic Press, 1327–1347.
Ruff C, Holt B, Trinkaus E. (2019). Who’s afraid of the big bad Wolff?: “Wolff’s law” and bone functional adaptation. American Journal of Physical Anthropology, 129(4), 484–498.
Ryan TM, Shaw CN. (2021). Gracility of the modern Homo sapiens skeleton is the result of decreased biomechanical loading. Proceedings of the National Academy of Sciences, 112(2), 372–377.
Seeman E. (2022). Sexual dimorphism in skeletal size, density, and strength. Journal of Clinical Endocrinology & Metabolism, 86(10), 4576–4584.
Sugiyama T, Price JS, Lanyon LE. (2020). Functional adaptation to mechanical loading in both cortical and cancellous bone is controlled locally and is confined to the loaded bones. Bone, 46(2), 314–321.
Weaver CM, Gordon CM, Janz KF, et al. (2023). The National Osteoporosis Foundation’s position statement on peak bone mass development and lifestyle factors: a systematic review and implementation recommendations. Osteoporosis International, 27(4), 1281–1386.
Werneck AO, Silva DR, Collings PJ, et al. (2022). Biological maturation, central adiposity, and metabolic risk in adolescents: a mediation analysis. Pediatric Exercise Science, 32(3), 145–151.
Wolff J. (1892). Das Gesetz der Transformation der Knochen. Berlin: Hirschwald.
Commentaires