Muscle Metamorphosis: The Science of Contractile Tissue Transformation
- Fitfty
- 2 days ago
- 14 min read
Updated: 9 hours ago
The Architectural Marvel Within: Understanding the Complex Organisation of Human Contractile Tissue
Article 3 of 4 in “The Strength Paradox” series

The human body contains a remarkable engine — a system of over 600 muscles constituting approximately 40% of total body mass and capable of generating forces that defy imagination. Yet despite our daily reliance on this extraordinary tissue, few truly understand the intricate architecture and molecular brilliance that transforms chemical energy into physical movement.
This article delves deep into the fascinating world of muscle physiology — from the nanoscale protein interactions that generate contractile force to the macroscopic organisation of muscle groups that determine athletic performance. By understanding the fundamental science of muscular development and function, athletes and coaches can unlock new dimensions of physical potential through targeted training approaches.
The Hierarchical Organisation of Contractile Tissue: From Whole to Molecular
Skeletal muscle exists as a meticulously organised hierarchical structure that spans multiple scales of biological organisation.
Macro-Level Architecture
At the most visible level, whole muscles anchor to bones via tendons, creating the fundamental mechanical system for movement. However, the external appearance of a muscle reveals little about its internal architecture — a critical determinant of function.
Muscles exhibit two primary architectural patterns:
Parallel Organisation: Muscle fibres run parallel to the line of pull, optimizing for range of motion and contraction velocity. Examples include the sartorius and rectus abdominis.
Pennate Organisation: Fibres attach obliquely to tendons at specific angles (the pennation angle), allowing more contractile tissue to attach to a limited tendon surface area. This arrangement maximizes force production at the expense of contraction velocity. The quadriceps and gastrocnemius exemplify this design.
The pennation angle — often described as part of the “muscle pentagon” concept — significantly influences performance capability. A larger pennation angle permits greater physiological cross-sectional area (PCSA) within a given volume, increasing potential force production. Elite power athletes typically display larger pennation angles in primary movers compared to untrained individuals.
The Muscle Fibre: The Functional Unit
Each muscle comprises thousands of individual muscle fibres — multinucleated cells ranging from 10–100μm in diameter and potentially spanning the entire length of the muscle. These remarkable cells represent the primary functional units of contraction.
Critical characteristics of muscle fibres include:
Length: Typically 2–30cm in humans, depending on the muscle
Diameter: Varies by fibre type and training status (10–100μm)
Nuclei: Multiple nuclei positioned peripherally along the sarcolemma
Innervation: Each fibre receives input from a single motor neuron at the neuromuscular junction
Adaptability: Capacity to hypertrophy, undergo fibre-type transition, and repair damage
The functional capacity of a fibre largely depends on its internal ultrastructure — an exquisitely organised array of contractile proteins arranged into repeating units.
Myofibrils and Sarcomeres: The Contractile Machinery
Within each muscle fibre exists numerous myofibrils — cylindrical organelles approximately 1–2μm in diameter running the length of the fibre. These contain the actual contractile apparatus arranged into functional units called sarcomeres.
The sarcomere represents the masterpiece of muscle architecture — a precisely organised assembly of interdigitating proteins:
Thin filaments: Primarily composed of actin, tropomyosin, and troponin complex
Thick filaments: Predominantly myosin molecules with protruding globular heads
Z-discs: Protein structures that anchor thin filaments and define sarcomere boundaries
M-line: Central region anchoring thick filaments
Titin: Massive elastic proteins spanning half-sarcomeres and providing structural integrity
This remarkable molecular machinery creates the distinctive banding pattern visible under electron microscopy:
A-band: Region containing thick filaments
I-band: Region containing only thin filaments
H-zone: Central region containing only thick filaments
Z-line: Boundary between adjacent sarcomeres
The precise alignment of these structures across adjacent myofibrils creates the striated appearance characteristic of skeletal muscle.
The Sliding Filament Theory: Understanding Muscle Contraction
The foundation of our understanding of muscle contraction lies in the sliding filament theory, first proposed independently by Hugh Huxley and Jean Hanson, and Andrew Huxley and Rolf Niedergerke in 1954. This elegant theory explains how muscles generate force through molecular interactions.
The Contractile Process
When a muscle contracts, the following sequence occurs:
Excitation: Action potentials from motor neurons trigger calcium release from the sarcoplasmic reticulum
Activation: Calcium binds to troponin C, causing conformational changes that expose myosin-binding sites on actin
Cross-bridge Formation: Energized myosin heads attach to exposed binding sites on actin
Power Stroke: Myosin heads pivot, pulling actin filaments toward the sarcomere center
Detachment: ATP binding causes myosin release from actin
Re-energisation: ATP hydrolysis re-cocks the myosin head for the next cycle
This cyclical process continues as long as calcium levels remain elevated and ATP remains available. Importantly, this mechanism explains a fundamental mechanical property of muscle: muscles can only pull, never push. All movement patterns — regardless of complexity — must be orchestrated through the coordinated contraction of agonists and relaxation of antagonists.
The sliding filament theory also elucidates key functional constraints:
Optimal length-tension relationship: Maximum force generation occurs at intermediate sarcomere lengths where actin-myosin overlap is optimal
Force-velocity relationship: As contraction velocity increases, force production decreases exponentially
Limited contraction range: Sarcomeres can only shorten to approximately 70% of their resting length
Understanding these constraints provides critical insights for training program design. For example, training muscles through full ranges of motion helps develop strength across varying muscle lengths, while velocity-specific training targets particular points along the force-velocity curve.
The Molecular Players: Proteins as Building Blocks
The extraordinary contractile capabilities of muscle result from specialised proteins evolutionarily refined for mechanical function.
Contractile Proteins
The primary force-generating interaction occurs between two key proteins:
Myosin: The thick filament protein comprising approximately 60% of muscle protein content. Each myosin molecule consists of:
Two heavy chains forming a coiled tail and globular heads
Four light chains (two regulatory, two essential) associated with each head
ATPase activity in the globular head region catalyzing energy conversion
Actin: Thin filament protein forming a double-stranded helix with binding sites for myosin. Individual globular actin monomers (G-actin) polymerise into filamentous actin (F-actin).
Regulatory Proteins
Several additional proteins control the contraction process:
Tropomyosin: Coiled-coil protein that wraps around the actin helix, blocking myosin-binding sites in the resting state
Troponin Complex:
Troponin C: Calcium-binding component
Troponin I: Inhibitory component
Troponin T: Tropomyosin-binding component
Structural Proteins
The sarcomere’s architecture depends on several structural proteins:
Titin: The largest protein in the human body, functioning as a molecular spring providing passive tension and sarcomere stability
Nebulin: Ruler protein regulating thin filament length
α-Actinin: Primary component of Z-discs
Myomesin: M-line protein anchoring thick filaments
These proteins work in concert to enable not only contraction but also the remarkable structural integrity necessary for muscle to withstand the tremendous forces it generates.
The Power Generator: Cross-Sectional Area and Force Production
A fundamental principle in muscle physiology is that maximum force production correlates directly with physiological cross-sectional area (PCSA) — the total area of all muscle fibres measured perpendicular to their long axes.
PCSA vs. Anatomical CSA
For parallel-fibred muscles, physiological and anatomical cross-sectional areas are approximately equal. However, for pennate muscles, PCSA exceeds anatomical CSA significantly. This explains why:
A pennate muscle can generate more force than a parallel muscle of equal volume
Experienced strength athletes develop pronounced pennation angles through hypertrophy
Short, thick muscles (high PCSA) typically excel in force production while long, slender muscles (low PCSA) excel in velocity and range
The relationship between PCSA and maximum force generation is remarkably consistent, with human skeletal muscle generating approximately 30–40 N/cm² regardless of the specific muscle. This mechanical constant forms the basis for theoretical models predicting human strength limits.
The Pentagon Model of Muscle Architecture
Modern biomechanical analysis often employs the “muscle pentagon” model to characterise the key architectural parameters determining muscle function:
Physiological cross-sectional area: Determining maximum force capacity
Fibre length: Influencing contractile velocity and excursion
Pennation angle: Affecting the geometric efficiency of force transmission
Moment arm: Determining the leverage effect of the force
Activation capacity: The neural drive’s ability to recruit available fibres
This integrated approach recognises that optimising muscle performance requires consideration of multiple architectural variables beyond simple size metrics.
The Spectrum of Power: Muscle Fibre Types
Not all muscle fibres are created equal. Human skeletal muscle contains a spectrum of fibre types with distinct contractile and metabolic properties.
Primary Classification
The traditional classification system identifies three main fibre types:
Type I (Slow Oxidative):
Slow contraction velocity
Low force production
High fatigue resistance
Rich mitochondrial content
Extensive capillary networks
Red appearance due to high myoglobin content
Primary energy system: Aerobic metabolism
2. Type IIa (Fast Oxidative Glycolytic):
Intermediate contractile velocity
Moderate force production
Moderate fatigue resistance
Substantial mitochondrial content
Good capillary supply
Red-pink appearance
Balanced use of aerobic and anaerobic systems
3. Type IIx (Fast Glycolytic):
Rapid contraction velocity
High force production
Low fatigue resistance
Limited mitochondrial content
Sparse capillary network
White appearance
Primary energy system: Anaerobic glycolysis
More sophisticated analysis reveals additional hybrid fibre types (e.g., Type IIax) representing intermediate properties along this continuum.
Molecular Basis of Fibre Type Differences
The contractile and metabolic properties of different fibre types result from variations in protein isoform expression:
Myosin Heavy Chain (MHC) Isoforms: Different MHC isoforms (I, IIa, IIx) exhibit varying ATPase activity rates, directly affecting contraction velocity
Metabolic Enzymes: Type I fibres express higher levels of oxidative enzymes, while Type II fibres express greater glycolytic enzyme content
Calcium-Handling Proteins: Variations in sarcoplasmic reticulum proteins affect calcium release and reuptake kinetics
Myoglobin Content: Higher in Type I fibres, enhancing oxygen storage and diffusion
These molecular differences translate into distinct functional capabilities, creating a spectrum of fibres optimised for different movement requirements — from postural maintenance to explosive power production.
Distribution and Trainability
Fibre type distribution varies significantly:
Between individuals: Genetic factors influence baseline distribution, with elite endurance athletes typically possessing higher Type I percentages and elite sprinters/power athletes higher Type II percentages
Between muscles: Postural muscles (e.g., soleus) contain predominantly Type I fibres, while high-power muscles (e.g., gastrocnemius) contain more Type II fibres
Within muscles: Most muscles contain mixed fibre types organized into motor units of similar types
While baseline fibre type distribution is largely genetically determined, training can induce limited fibre type transitions, primarily between Type IIx and Type IIa. Endurance training typically promotes conversion of Type IIx to Type IIa, while detraining or explosive training can induce Type IIa to Type IIx transition. Conversion between Type II and Type I fibres occurs to a much more limited extent, explaining why elite performance in opposite ends of the power-endurance spectrum likely requires appropriate genetic predisposition.
The Control System: Neural Regulation of Muscle
Muscle architecture and fibre composition represent only part of the strength equation. The nervous system’s ability to activate and coordinate muscle fibres plays an equally crucial role.
Motor Units: The Functional Neural-Muscular Unit
A motor unit consists of:
A single alpha motor neuron in the spinal cord
All muscle fibres innervated by that neuron (ranging from a few fibres in precisely controlled muscles to thousands in large power muscles)
Motor units follow the “all-or-none” principle — when activated, all fibres in the unit contract simultaneously. However, the nervous system precisely controls force production through two primary mechanisms:
Recruitment: Activating increasing numbers of motor units
Rate Coding: Increasing the firing frequency of active motor units
These mechanisms follow predictable patterns:
Size Principle: Smaller motor units (typically Type I) are recruited first, with progressively larger units (Type II) activated as force requirements increase
Reverse Decruitment: Motor units are generally deactivated in reverse order of recruitment
Asynchronous Recruitment: Under submaximal conditions, motor units activate and deactivate in cycling patterns to prevent fatigue
Skilled motor performance depends on the precise orchestration of these mechanisms across multiple muscles.
Satellite Cells: The Regenerative Reserve
A critical yet often overlooked component of muscle tissue is the satellite cell population — muscle-specific stem cells residing between the sarcolemma and basal lamina of muscle fibres. These cells remain quiescent under normal conditions but activate in response to damage or growth stimuli.
Satellite cells serve several essential functions:
Hypertrophic Support: Donating nuclei to growing muscle fibres, maintaining the nuclear domain (cytoplasmic volume served by each nucleus)
Regeneration: Proliferating and differentiating to replace damaged fibres
Remodeling: Facilitating architectural adaptations to changing functional demands
The satellite cell response varies with:
Age: Declining in number and activation capacity with advancing age
Fibre Type: Type I fibres typically possess more satellite cells than Type II
Training Status: Resistance training increases satellite cell content
Hormonal Environment: Testosterone and growth factors enhance activation
Recent research suggests satellite cell activity may represent a limiting factor in extreme hypertrophy, particularly in advanced trainees. This has profound implications for long-term periodisation approaches and recovery strategies.
The Force-Velocity-Power Relationship: The Mechanical Paradigm
Understanding muscle mechanics requires appreciation of three interrelated variables: force, velocity, and power.
The Force-Velocity Curve
As contraction velocity increases, force production capacity decreases following a hyperbolic relationship:
Maximum force occurs during isometric or very slow eccentric contractions
Force decreases non-linearly as concentric velocity increases
At maximum contractile velocity, force approaches zero
This relationship emerges directly from the cross-bridge cycling mechanism — faster movements allow less time for cross-bridge formation and force development.
The Power Curve
Power — the product of force and velocity — follows a parabolic relationship:
Zero power at isometric conditions (velocity = 0)
Zero power at maximum velocity (force = 0)
Peak power at intermediate velocities (typically ~30–40% of maximum contractile velocity)
This relationship explains why:
Different sporting movements occur at different points on the force-velocity spectrum
Training specificity matters tremendously for performance optimization
Mixed-methods training approaches typically produce superior results to single-methodology programs
The Length-Tension Relationship and Optimal Contraction Level
Muscle generates different forces at different lengths due to varying degrees of actin-myosin overlap:
Maximum force occurs at optimal length (slight stretch beyond resting length)
Force decreases at shorter lengths due to:
Actin filament overlap from opposite sides of the sarcomere
Interference from compressed structural elements
Suboptimal myofilament spacing
Force also decreases at longer lengths due to:
Reduced actin-myosin overlap
Suboptimal cross-bridge angle
Eventually, complete loss of overlap
This relationship creates an “optimal contraction level” for each movement — a specific range of motion where force production capabilities are maximized. Elite lifters and coaches intuitively understand this principle, focusing on the “sticking point” of movements where mechanical disadvantage is greatest.
Metabolic Engines: Energy Systems and Muscle Function
Muscle contraction requires energy in the form of ATP, which must be continuously regenerated through integrated metabolic pathways.
The ATP-PC System: Immediate Energy
The phosphocreatine system provides immediate ATP replenishment:
Creatine phosphate donates phosphate to ADP, rapidly regenerating ATP
Powers maximum efforts lasting 5–10 seconds
Limited by intramuscular creatine phosphate stores
Recovery requires 3–5 minutes for complete restoration
Enhanced through creatine supplementation and high-intensity training
Glycolytic System: Short-Term Energy
Anaerobic glycolysis provides rapid but limited ATP production:
Converts glucose/glycogen to lactate without oxygen
Predominates in efforts lasting 30–90 seconds
Limited by pH decline and enzyme inhibition
Recovery requires 15–30 minutes for waste product clearance
Enhanced through high-intensity interval training
Oxidative System: Sustained Energy
Aerobic metabolism provides sustainable but slower ATP regeneration:
Complete oxidation of carbohydrates, fats, and (minimally) proteins
Predominates in efforts beyond 2–3 minutes
Limited primarily by oxygen delivery and mitochondrial density
Enhanced through endurance training increasing capillarisation and mitochondrial biogenesis
Energy System Interaction and Recovery
All three systems operate simultaneously, with varying contributions depending on effort intensity and duration. Recovery processes include:
Immediate recovery: Replenishment of ATP and phosphocreatine stores
Short-term recovery: Lactate clearance and glycogen restoration
Long-term recovery: Protein synthesis and structural repair
The interaction between these systems creates the physiological basis for periodisation models targeting specific adaptations through carefully programmed training stimuli.
Epigenetic Influences: Environmental Modulation of Muscle Expression
Muscle exhibits remarkable plasticity in response to environmental stimuli through epigenetic mechanisms — changes in gene expression without alteration of the underlying DNA sequence.
Key Epigenetic Mechanisms
Several processes regulate muscle gene expression:
DNA Methylation: Addition of methyl groups to DNA, typically suppressing gene expression
Histone Modification: Chemical alterations to histone proteins affecting chromatin structure
MicroRNAs: Small non-coding RNAs regulating mRNA stability and translation
These mechanisms mediate the muscle’s response to:
Mechanical loading (resistance training)
Metabolic stress (endurance training)
Nutritional factors
Hormonal signals
Inflammatory processes
Age-related changes
Training-Induced Epigenetic Adaptations
Different training modalities induce distinct epigenetic signatures:
Resistance Training: Triggers hypomethylation of genes involved in hypertrophy pathways, including myogenic regulatory factors, growth factor receptors, and protein synthesis regulators
Endurance Training: Promotes modifications enhancing expression of mitochondrial proteins, metabolic enzymes, and capillarization factors
Inactivity/Immobilization: Induces hypermethylation of metabolic and structural genes, suppressing muscle maintenance pathways
Perhaps most fascinatingly, some training adaptations create “epigenetic memory” — facilitating more rapid readaptation following detraining periods. This phenomenon partially explains both the concept of “muscle memory” and the potential long-term advantages gained from early-life physical activity.
Practical Applications: Translating Science to Performance
Understanding muscle architecture and physiology provides the foundation for evidence-based training approaches.
Training for Architectural Adaptation
To optimise muscle architecture for specific performance goals:
For maximum force production: High-load training (80–100% 1RM) with emphasis on compound movements increases physiological cross-sectional area and pennation angle
For explosive power: Combined heavy and ballistic training develops both cross-sectional area and neural activation capacity while maintaining optimal pennation angles for force transfer
For endurance capacity: Higher-volume, moderate-load training enhances capillarisation while maintaining appropriate architectural properties
Fibre Type-Specific Training
Different protocols preferentially target specific fibre types:
Type I enhancement: Sustained submaximal contractions, blood flow restriction training with light loads, endurance-focused protocols
Type IIa development: Moderate-load, moderate-volume training with limited rest periods (e.g., traditional bodybuilding approaches)
Type IIx preservation/development: Very high-load, low-volume training with extended rest periods, explosive movements, and appropriate detraining phases
Recovery Optimisation
Understanding the energetic and structural bases of fatigue informs recovery strategies:
Phosphocreatine restoration: 3–5 minute rest periods between maximum effort sets
Glycogen replenishment: Carbohydrate intake timed with the post-exercise “window of opportunity”
Structural repair: Protein provision supporting myofibrillar protein synthesis
Satellite cell activation: Appropriate hormonal environment through nutrition, rest, and stress management
Conclusion: The Transformative Potential of Muscular Understanding
The science of muscle metamorphosis reveals a tissue of extraordinary complexity and adaptability — capable of transforming in structure and function in response to specific demands. From the molecular ballet of the sliding filament theory to the architectural brilliance of pennate designs, muscle tissue represents one of nature’s most remarkable achievements in biological engineering.
For athletes and coaches, this knowledge transcends academic interest, providing the theoretical foundation for truly optimized training approaches. By understanding the structural and functional properties of muscle — from cross-sectional area to fibre type distribution to energetic systems — we can design interventions that specifically target limiting factors in performance.
Perhaps most importantly, this understanding reveals the remarkable plasticity of human physical capability. While genetic factors unquestionably influence baseline characteristics and ultimate potential, the adaptive capacity of muscle tissue provides tremendous scope for transformation. Through precisely applied stimuli and carefully structured progression, the human muscular system can undergo metamorphosis from untrained to extraordinary — a testament to our evolutionary design and the power of applied exercise science.
Next Up:
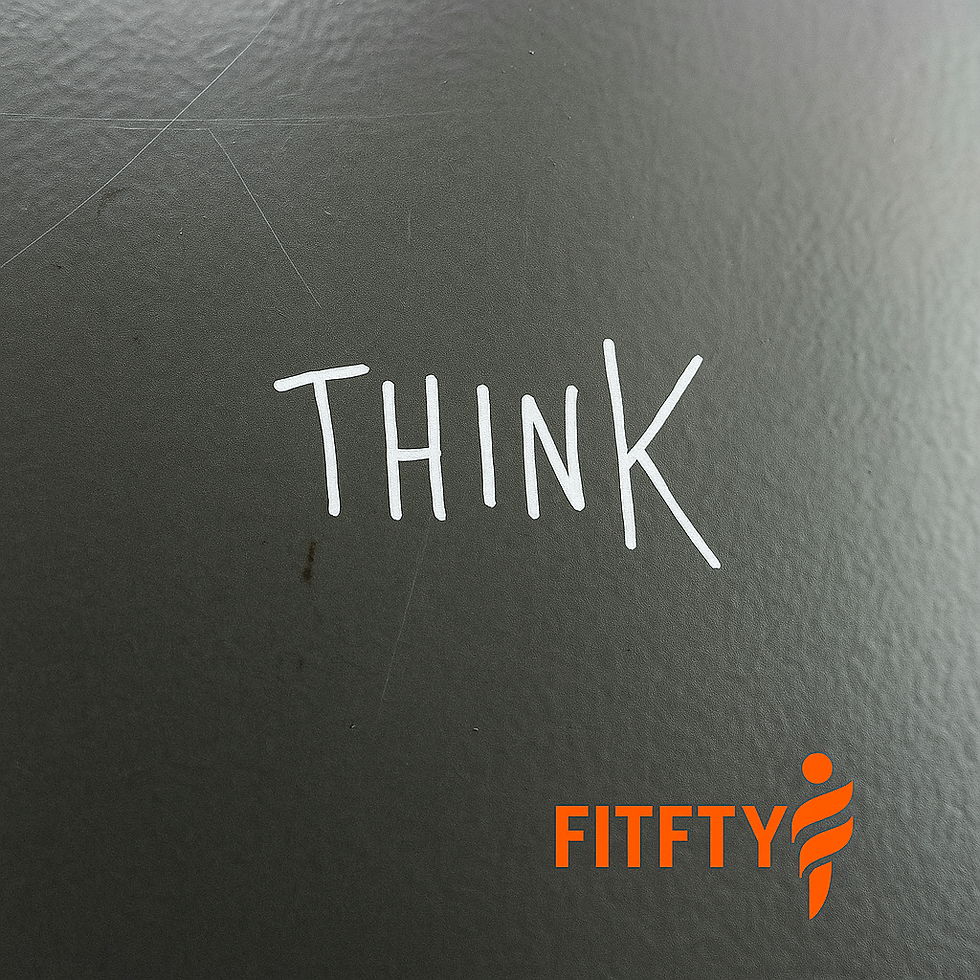
🔍 Explore More from Fitfty
Because strength isn’t a moment — it’s a movement.
🧱The Prerequisites for Strength
9-Part Series | Get strength-ready from the ground up
Joints, bones, tissues, hormones, nutrition, mindset — this series is the blueprint your body’s been waiting for.
💥 You don’t build strength by accident. You build it by design.
🧠 More Than Muscle: What Mortality, Setbacks, and Superhumans Reveal
Mini-Series | 3 stories. 3 truths. One very human journey.
Strength is personal. It’s shaped by loss, built through adversity, and tested by time. This series digs into the why behind the weight — and the people who carry it.
🔥 You won’t see strength the same way again.
Series Menu:
The Strength Paradox: Why Everything You Know About Building Your Body is About to Change
3 — Muscle Metamorphosis: The Science of Contractile Tissue Transformation
📚 References
Aagaard P, Andersen JL. (2022). Effects of strength training on muscle fiber types and size; consequences for athletes training for high-intensity sport. Scandinavian Journal of Medicine & Science in Sports, 20(Suppl 2), 32–38.
Bottinelli R, Reggiani C. (2020). Human skeletal muscle fibres: molecular and functional diversity. Progress in Biophysics and Molecular Biology, 73(2–4), 195–262.
Close RI. (1972). Dynamic properties of mammalian skeletal muscles. Physiological Reviews, 52(1), 129–197.
Folland JP, Williams AG. (2023). The adaptations to strength training: morphological and neurological contributions to increased strength. Sports Medicine, 37(2), 145–168.
Huxley AF, Niedergerke R. (1954). Structural changes in muscle during contraction; interference microscopy of living muscle fibres. Nature, 173(4412), 971–973.
Huxley H, Hanson J. (1954). Changes in the cross-striations of muscle during contraction and stretch and their structural interpretation. Nature, 173(4412), 973–976.
Jones DA, Rutherford OM, Parker DF. (1989). Physiological changes in skeletal muscle as a result of strength training. Quarterly Journal of Experimental Physiology, 74(3), 233–256.
Komi PV. (2008). Stretch-shortening cycle: a powerful model to study normal and fatigued muscle. Journal of Biomechanics, 33(10), 1197–1206.
Lieber RL, Fridén J. (2019). Functional and clinical significance of skeletal muscle architecture. Muscle & Nerve, 23(11), 1647–1666.
Mauro A. (1961). Satellite cell of skeletal muscle fibers. The Journal of Biophysical and Biochemical Cytology, 9(2), 493–495.
Merton PA. (1954). Voluntary strength and fatigue. The Journal of Physiology, 123(3), 553–564.
Pette D, Staron RS. (2000). Myosin isoforms, muscle fiber types, and transitions. Microscopy Research and Technique, 50(6), 500–509.
Schiaffino S, Reggiani C. (2023). Fiber types in mammalian skeletal muscles. Physiological Reviews, 91(4), 1447–1531.
Seynnes OR, de Boer M, Narici MV. (2021). Early skeletal muscle hypertrophy and architectural changes in response to high-intensity resistance training. Journal of Applied Physiology, 102(1), 368–373.
van der Zwaard S, Weide G, Levels K, et al. (2023). Muscle architectural changes in elite athletes after high-impact and high-volume training. Journal of Science and Medicine in Sport, 22(4), 411–416.
Proof of Autorship:
This article was signed by the author using Keybase.
View the signed proof here.
Kommentit